Epigenetic Memory: Binary or Analog?
![<strong>Figure 1.</strong> Foundations of epigenetic memory. <strong>1a.</strong> The same genotype results in different gene expression states that are maintained across many cell divisions. Histone 3 lysine 9 trimethylation (H3K9me3) and DNA methylation (DNAme) preserve the silenced gene state (OFF), and histone 3 lysine 4 trimethylation (H3K4me3) is associated with the active gene state (ON). <strong>1b.</strong> Conrad Waddington’s epigenetic landscape [9]. Pluripotent stem cells are at the top of the hill, while the different valleys at the bottom of the hill indicate distinct cell fates. <strong>1c.</strong> Histone modification circuit that includes unmodified histones, histones with H3K9me3 modification, and histones with H3K4me3 modification [3]. <strong>1d.</strong> Steady state landscape wherein open circles signify unstable steady states and closed circles signify stable steady states [3]. Figures 1a, 1c, and 1d courtesy of the author; Figure 1b courtesy of [9].](/media/tnqpr15c/figure1.jpg)
Through epigenetic memory, cells are able to temporally maintain multiple gene expression states without changes to their genetic sequence (see Figure 1a). One can visualize this critical property in the context of cellular differentiation with Conrad Waddington’s well-known epigenetic landscape [9]; according to this metaphor, cells make binary decisions as genes become activated or repressed while the cell travels downhill through a landscape, wherein each valley represents a different cell type (see Figure 1b). Because the gene expression pattern that corresponds to each of these types remains “frozen” in time, it prevents unwanted conversions between various cell types that would compromise the organism’s health. But how is this possible?
Chromatin modifications—i.e., chemical modifications to DNA and histones—are thought to be major mediators for the long-term maintenance of gene expression states. For example, histone 3 lysine 9 trimethylation (H3K9me3) and DNA methylation (DNAme) enable the long-term maintenance of completely silenced gene states [5], while histone 3 lysine 4 trimethylation (H3K4me3) is associated with active gene states [6] (see Figure 1a). Such modifications modulate transcription by affecting chromatin compaction; they propagate through DNA replication and cell division via maintenance processes [5, 7].
For DNAme, these maintenance processes involve an enzyme called DNA-methyltransferase 1 (DNMT1) that quickly copies the exact methylation pattern of the mother DNA strand onto the daughter strand, thereby compensating for the mark’s dilution rate and conferring long-term stability [7]. In contrast, histone modifications are maintained because they consist of autocatalytic enzymatic reactions, in which a modified histone binds to “reader” proteins that recruit writers of the same mark [5]. These two processes create a positive feedback loop that may induce bistability, wherein a gene can have at most two stable steady states: unmodified or fully modified [3, 4] (see Figures 1c and 1d). Consistent with this binary memory model, experimental studies with a chromosomally integrated reporter gene have shown that histone modifications mediate an epigenetic switch where gene expression is either switched “on” or “off” [1].
Mathematical Modeling for Epigenetic Memory
Using a mathematical model, we investigated whether additional forms of memory (beyond binary memory) are realizable with chromatin modifications. Other possible forms of memory include n-ary memory—in which \(n>2\) stable states are possible—and analog memory, which can stably maintain each state. While the former arises from multistable systems wherein each stable state is locally attractive, the latter cannot emerge from multistability because its stable states are not locally attractive. The histone modification circuit model that dominates the current understanding of epigenetic memory is either bistable (binary memory) or monostable (no memory) and hence cannot reproduce a situation of n-ary or analog memory (see Figures 1c and 1d).
However, histone modifications alone do not capture the entirety of the chemical modifications that control the state of chromatin for genes in the mammalian chromosome. DNAme is also present, and histone modifications operate not in isolation but rather in orchestration with DNAme. Specifically, DNAme acts cooperatively with the repressive modification of H3K9me3 by recruiting writers of the latter; H3K9me3 can also recruit writers of DNAme, thereby creating a positive feedback loop. Similarly, DNAme acts competitively with H3K4me3 since these two marks recruit each other’s erasers. When combined with the histone modification reaction model in Figure 1c, these “crosstalks” yield a much richer set of chemical reactions that we term the chromatin modification circuit [3] (see Figure 2a).
![<strong>Figure 2.</strong> The transition between binary and analog epigenetic memory. <strong>2a.</strong> Chromatin modification circuit that includes both histone modifications—specifically, histone 3 lysine 9 trimethylation (H3K9me3) and histone 3 lysine 4 trimethylation (H3K4me3)—and DNA methylation (DNAme). DNAme is established by writer DNA methyltransferase 3A (DNMT3A) and “erased” by enzyme ten-eleven translocation methylcytosine dioxygenase 1 (TET1); otherwise, it is essentially conserved as the maintenance action of DNMT1 compensates for the dilution process. The emergent decay rate constant for DNAme is \(\mu\). DNAme recruits writers of H3K9me3 (red dashed arrows) and erasers of H3K4me3 (grey dashed arrows marked by \(\varepsilon'\)). Similarly, H3K9me3 recruits writers of DNAme (yellow dashed arrows marked by \(\alpha'\)) while H3K4me3 recruits TET1 (blue dashed arrow marked by \(\varepsilon'\)). <strong>2b.</strong> The transition between analog and binary memory. On the left, the positive feedback between DNAme and H3K9me3 is broken. Any initial DNAme grades and their corresponding gene expression levels remain approximately constant in time. On the right, there is positive feedback between DNAme and H3K9me3. Any initial DNAme grades converge to either full DNAme (no gene expression) or no DNAme (maximal gene expression). <strong>2c.</strong> Binary and analog memory. Figure courtesy of [8].](/media/w5ohacai/figure2.jpg)
Although this model may seem intricate, we can use it to build an intuition for the chromatin modification state’s expected long-term behavior. We start with a small level of H3K9me3 (or DNAme), which recruits writers for DNAme (or H3K9me3), which in turn recruits writers for H3K9me3 (or DNAme) — ultimately leading to a buildup of repressive chemical modifications that fully silences gene expression. But if the initial level of repressive modifications is too low, then they will not be able to enhance the removal of H3K4me3; the mark will consequently build up via its positive feedback and cause full gene expression. This qualitative reasoning is in accordance with mathematical analysis of the steady state behavior, which indicates bistability as long as the positive feedback on both repressive and activating marks—as well as the mutual inhibition between these opposing marks—is sufficiently strong [3].
The chromatin modification circuit model in Figure 2a includes interactions that have been experimentally documented. But since chromatin modifications are context-dependent, it is possible that not all reactions are equally strong in every genetic and cellular context. For example, H3K9me3 does not recruit writers of DNAme in certain situations [1, 8]. We therefore studied the system’s behavior with weaker positive feedback between H3K9me3 and DNAme by decreasing the rate \(\alpha'\) at which H3K9me3 recruits writers of DNAme (see Figure 2a). Stochastic simulations of the full chemical reaction system show that as \(\alpha'\) approaches zero, it takes increasingly long for any initial DNAme grade in the gene to appreciably change. Instead, histone modifications quickly change around a mean value that is determined by the DNAme grade, as does gene expression (see Figure 2b). That is, memory of gene expression changes from binary to analog as \(\alpha'\) approaches zero (see Figure 2c).
We can qualitatively explain this outcome as follows. The reactions that alter the DNAme grade in the absence of external input (the writer DNMT3A enzyme) are only given by (i) the establishment of DNAme due to H3K9me3’s recruitment of writer enzymes (encoded by \(\alpha'\)) and (ii) the decay rate due to dilution and “erasure” enzyme ten-eleven translocation methylcytosine dioxygenase 1 (TET1). In the absence of erasure enzymes, and assuming a highly efficient maintenance process by DNMT1, the decay rate of DNAme is practically zero. Therefore, the only change to the DNAme state is due to the rate \(\alpha'\). As \(\alpha'\) approaches zero, the DNAme grade will thus remain constant over time.
New Model Predicts Analog Memory
![<strong>Figure 3.</strong> Analog epigenetic memory emerges when the positive feedback loop between DNAme and H3K9me3 is broken. <strong>3a.</strong> Simplified chromatin modification circuit where \(\alpha'=0\) and ten-eleven translocation methylcytosine dioxygenase 1 (TET1) \(=0\). A temporal pulse of the DNA methylation (DNAme) writer—i.e., DNA methyltransferase 3A (DNMT3A)—can set the total DNAme grade. <strong>3b.</strong> Stationary behavior of the chromatin modification circuit in Figure 3a with parameter values from [8]. Figure courtesy of the author and [8].](/media/e21ip14f/figure3.jpg)
When \(\alpha'\) and the decay rate of DNAme \(\mu\) are both set to zero, the chromatin modification circuit simplifies to what is shown in Figure 3a. This chemical reaction model well approximates the full system as \(\alpha\) and \(\mu\) approach zero. Mathematically, we can demonstrate this fact through a regular perturbation analysis of the ordinary differential equation system that describes the full chemical modification circuit, and by taking the Taylor expansion of the solution for small \(\alpha\) and \(\mu\) [2]. The reduced chemical reaction circuit reveals that DNAme remains frozen in time at its initial condition, and the histone modification circuit essentially gives the leftover dynamics. In other words, the DNAme grade appears as an input that “drives” the histone modification dynamics by modulating the mean value about which H3K9me3 and H3K4me3 hover. The histone modification circuit can be bistable (with sufficiently small \(\epsilon\)) for intermediate values of the DNAme grade, while the circuit is monostable for low and high values of DNAme. This change of stability—under the influence of noise—produces a probability distribution of gene expression that broadens significantly for intermediate DNAme grades (see Figure 3b). It ultimately leads to more variability of gene expression when the gene is partially methylated, which is consistent with experimental evidence [8]. In summary, our model predicts that (i) analog memory of gene expression is encoded in the DNAme grade, and (ii) histone modifications only “track” the DNAme grade and are responsible for gene expression variability.
To conclude, our chemical reaction model of chromatin modifications at genes of the mammalian chromosome explores different possible regimes of epigenetic memory: binary or analog. The model explains the transition between these two regimes under varying strength of the positive feedback between DNAme and repressive histone modifications. Analog memory emerges when this feedback is weak, while strong positive feedback leads to binary memory. Because chromatin modifications and their crosstalks are highly dependent on context—as are the values of chemical parameters—we expect different genetic and cellular settings to display different types of memory. While analog memory may be possible in some cell types, others may only support binary memory. From an engineering standpoint, the ability to control memory type and program analog memory by graded DNAme will significantly impact a variety of applications in synthetic biology, gene and cell therapy, and cell and tissue engineering.
Domitilla Del Vecchio delivered an invited presentation on this research at the 2024 SIAM Conference on the Life Sciences, which took place in Portland, Ore., last June.
References
[1] Bintu, L., Yong, J., Antebi, Y.E., McCue, K., Kazuki, Y., Uno, N., … Elowitz, M.B. (2016). Dynamics of epigenetic regulation at the single-cell level. Science, 351(6274), 720-724.
[2] Bruno, S., & Del Vecchio, D. (2024). Epigenetic cell memory: Binary or analog? In Proceedings of the 2024 IEEE 63rd conference on decision and control (pp. 7764-7771). Milan, Italy: Institute of Electrical and Electronics Engineers.
[3] Bruno, S., Williams, R.J., & Del Vecchio, D. (2022). Epigenetic cell memory: The gene’s inner chromatin modification circuit. PLoS Comput. Biol., 18(4), e1009961.
[4] Dodd, I.B., Micheelsen, M.A., Sneppen, K., & Thon, G. (2007). Theoretical analysis of epigenetic cell memory by nucleosome modification. Cell, 129(4), 813-822.
[5] Hathaway, N.A., Bell, O., Hodges, C., Miller, E.L., Neel, D.S., & Crabtree, G.R. (2012). Dynamics and memory of heterochromatin in living cells. Cell, 149(7), 1447-1460.
[6] Huisinga, K.L., Brower-Toland, B., & Elgin, S.C.R. (2006). The contradictory definitions of heterochromatin: Transcription and silencing. Chromosoma, 115(2) 110-122.
[7] Li, E., & Zhang, Y. (2014). DNA methylation in mammals. Cold Spring Harb. Perspect. Biol., 6(5), a019133.
[8] Palacios, S., Bruno, S., Weiss, R., Salibi, E., Kane, A., Ilia, K., & Del Vecchio, D. (2024). Analog epigenetic cell memory by graded DNA methylation. Preprint, bioRxiv.
[9] Waddington, C.H. (1957). The strategy of the genes. London, England: Routledge.
About the Author
Domitilla Del Vecchio
Professor, Massachusetts Institute of Technology
Domitilla Del Vecchio is a professor of mechanical and biological engineering at the Massachusetts Institute of Technology. Her research interests lie in the use and development of control and dynamical systems tools for the analysis and engineering of living systems.
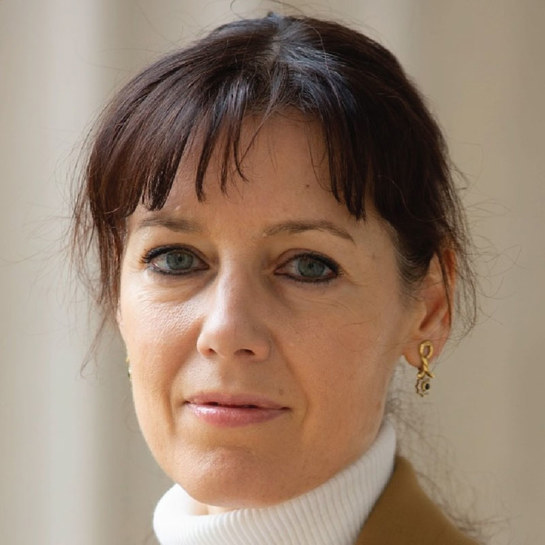
Stay Up-to-Date with Email Alerts
Sign up for our monthly newsletter and emails about other topics of your choosing.